Green energy technologies for combating climate change
Green energy technologies for combating climate change are rapidly evolving, offering a crucial pathway to mitigate the devastating effects of global warming. This exploration delves into the diverse array of renewable energy sources – solar, wind, hydro, geothermal, and biomass – examining their technological advancements, environmental impacts, and economic implications. We will also investigate the crucial role of energy storage and smart grid technologies in facilitating a seamless transition to a sustainable energy future, considering the policy frameworks and future trends shaping this critical global endeavor.
The urgency of climate action necessitates a comprehensive understanding of these technologies, their potential benefits, and the challenges associated with their widespread adoption. This discussion aims to provide a balanced perspective, acknowledging both the immense promise and the inherent complexities involved in transitioning to a cleaner, more sustainable energy system.
Introduction to Green Energy Technologies
The escalating global climate crisis demands immediate and decisive action. The scientific consensus overwhelmingly points to the urgent need to drastically reduce greenhouse gas emissions to mitigate the worst effects of climate change. Technological advancements in green energy offer a crucial pathway towards achieving this goal, providing sustainable alternatives to fossil fuels and paving the way for a cleaner, more resilient future.
Delaying the widespread adoption of these technologies will only exacerbate the challenges we face.Green energy technologies encompass a diverse range of methods for generating electricity and heat from renewable and sustainable sources. These technologies offer a compelling alternative to traditional energy sources that rely heavily on fossil fuels, which are major contributors to climate change. The transition to green energy is not simply about replacing one energy source with another; it’s about fundamentally reshaping our energy systems to be more sustainable, efficient, and resilient.
Overview of Green Energy Technologies
Several key technologies are driving the global shift towards a greener energy landscape. Solar power, harnessing the sun’s energy through photovoltaic cells or concentrated solar power systems, is a rapidly expanding sector. Wind power, utilizing wind turbines to convert kinetic energy into electricity, offers a reliable source of energy, particularly in areas with consistent wind patterns. Hydropower, leveraging the energy of flowing water to generate electricity, has been a mainstay of renewable energy for decades, although its expansion is often constrained by environmental concerns.
Geothermal energy taps into the Earth’s internal heat to produce electricity and heat, offering a stable and consistent energy source. Bioenergy utilizes biomass, organic matter from plants and animals, to generate electricity or biofuels. Finally, emerging technologies like tidal and wave energy are also showing promise, although they are still in earlier stages of development and deployment.
Potential Impact on Global Carbon Emissions
Widespread adoption of green energy technologies has the potential to significantly reduce global carbon emissions. For example, a complete transition to renewable energy sources in the electricity sector could dramatically decrease carbon dioxide emissions associated with power generation. The International Renewable Energy Agency (IRENA) has modeled various scenarios, showing that a rapid scaling-up of renewable energy could significantly reduce global emissions by mid-century.
These models often demonstrate that a transition to renewable energy is not only environmentally beneficial but also economically viable, creating jobs and stimulating economic growth. The success of this transition, however, hinges on overcoming challenges related to infrastructure development, energy storage, and policy support. Real-world examples, such as Denmark’s significant progress in wind energy integration and Germany’s Energiewende initiative (energy transition), illustrate the potential for substantial reductions in carbon emissions through large-scale green energy deployment.
These initiatives, while facing their own challenges, demonstrate the feasibility of ambitious climate goals through technological advancements and policy changes.
Solar Energy Technologies
Harnessing the sun’s energy offers a significant pathway towards a sustainable future. Solar energy technologies provide a clean and renewable source of power, playing a crucial role in mitigating climate change. These technologies are constantly evolving, leading to increased efficiency and reduced costs, making them increasingly competitive with traditional energy sources.
Solar energy technologies are broadly categorized into two main types: photovoltaic (PV) and concentrated solar power (CSP). Both leverage the sun’s radiant energy, but they differ significantly in their methods of energy conversion and application.
Photovoltaic Solar Energy
Photovoltaic (PV) technology converts sunlight directly into electricity using semiconductor materials, typically silicon. Sunlight striking the PV cell generates an electric current through the photovoltaic effect. These cells are assembled into modules, which are then combined to form larger arrays or solar panels. Different PV technologies exist, each with its own advantages and disadvantages in terms of efficiency, cost, and durability.
For instance, monocrystalline silicon cells offer higher efficiency but are more expensive to produce compared to polycrystalline silicon cells, which have slightly lower efficiency but are more cost-effective. Thin-film solar cells, using materials like cadmium telluride or copper indium gallium selenide (CIGS), offer flexibility and lighter weight but generally have lower efficiency.
Concentrated Solar Power
Concentrated solar power (CSP) systems utilize mirrors or lenses to concentrate sunlight onto a receiver, generating heat that drives a heat engine (typically a steam turbine) to produce electricity. There are several types of CSP systems, including parabolic trough, power tower, and linear Fresnel reflector systems. These systems are particularly suitable for large-scale electricity generation, offering the advantage of energy storage through the use of molten salt or other thermal storage mediums.
This allows for electricity generation even after sunset, addressing the intermittency issue associated with solar energy.
Comparison of Solar Panel Technologies
Choosing the most appropriate solar technology depends on various factors, including cost, efficiency, available space, and environmental impact. The following table summarizes the pros and cons of different solar technologies:
Technology | Pros | Cons | Efficiency Range |
---|---|---|---|
Monocrystalline Silicon | High efficiency, aesthetically pleasing, long lifespan | High cost, requires significant land area for large-scale deployment | 18-22% |
Polycrystalline Silicon | Lower cost than monocrystalline, readily available | Lower efficiency than monocrystalline, less aesthetically pleasing | 15-17% |
Thin-Film (CdTe, CIGS) | Flexible, lightweight, suitable for integration into building materials | Lower efficiency, potential environmental concerns regarding material composition | 8-12% |
Concentrated Solar Power (CSP) | High efficiency potential, energy storage capability | High initial cost, requires large land area, water usage for cooling | Up to 35% (with energy storage) |
Challenges and Opportunities in Large-Scale Solar Deployment
Large-scale solar energy deployment faces several challenges, including land use requirements, grid integration complexities, intermittency of solar resource, and the need for efficient energy storage solutions. However, significant opportunities exist. Advances in technology are continually improving efficiency and reducing costs. Government policies and incentives are promoting solar energy adoption, while decreasing costs and improving grid infrastructure are further enabling large-scale deployments.
For example, the significant growth of solar energy in countries like China and India demonstrates the potential for large-scale implementation, despite the initial challenges. Furthermore, the integration of solar energy with other renewable energy sources, such as wind power, can create more resilient and reliable energy systems. The development of advanced energy storage technologies, such as battery storage and pumped hydro storage, is also crucial for addressing the intermittency issue and enabling a greater reliance on solar power.
Wind Energy Technologies
Wind energy harnesses the kinetic energy of moving air to generate electricity. This technology plays a significant role in the global transition to renewable energy sources, offering a clean and sustainable alternative to fossil fuels. The efficiency and scale of wind energy generation have increased dramatically in recent years, driven by technological advancements and increasing demand for renewable energy.
Wind turbines, the heart of wind energy systems, come in various designs and sizes, categorized primarily as onshore and offshore installations. Each type presents unique technological challenges and opportunities, influencing their environmental impact and economic viability.
Onshore and Offshore Wind Turbine Technologies
Onshore wind turbines are typically erected on land, often in areas with consistent and strong winds, such as plains, hills, or coastal regions. These turbines are generally smaller and less powerful than their offshore counterparts, but their construction and maintenance are comparatively simpler and less expensive. They utilize various technologies, including horizontal-axis wind turbines (HAWTs), which are the most common type, featuring a horizontal rotor axis and a tower supporting the nacelle containing the generator.
Vertical-axis wind turbines (VAWTs) are less prevalent but offer advantages in certain applications due to their ability to capture wind from multiple directions.
Offshore wind turbines, installed in bodies of water, are larger and more powerful than onshore turbines. This is due to higher and more consistent wind speeds over water. Their construction and maintenance are significantly more complex and costly, requiring specialized vessels and equipment. However, the higher energy yields compensate for the increased investment. Offshore wind farms are typically located further from shore to maximize wind resources and minimize visual impact on coastal communities.
Floating offshore wind turbines are a developing technology, particularly suitable for deeper waters where fixed-bottom structures are impractical.
Environmental Impacts of Wind Energy Projects and Mitigation Strategies
While wind energy is a clean energy source, it’s not without environmental impacts. These include habitat disruption from construction and land use changes (for onshore projects), potential harm to birds and bats (collision with turbine blades), and noise pollution. Offshore wind farms may affect marine ecosystems, potentially impacting fish populations and marine mammals.
Mitigation strategies aim to minimize these negative effects. Careful site selection, using environmental impact assessments to identify sensitive areas and avoid them, and implementing bird and bat deterrent systems on turbines are crucial. For offshore projects, environmental monitoring programs can help assess and manage potential impacts on marine life. Noise reduction technologies are also employed to minimize the impact on nearby communities.
Proper decommissioning plans are essential to minimize the long-term environmental footprint of wind farms when they reach the end of their operational life.
Hypothetical Wind Farm Design
This hypothetical wind farm, named “Seabreeze Wind,” will be located offshore in the North Sea, approximately 50 kilometers off the coast of a suitable location (e.g., a region with consistent high wind speeds and minimal environmental sensitivities after careful assessment). The farm will consist of 100 large-capacity offshore wind turbines, each with a rated capacity of 15 megawatts (MW), resulting in a total installed capacity of 1500 MW.
Environmental considerations will be central to the project’s design and operation. A comprehensive environmental impact assessment will be conducted to identify and mitigate potential impacts on marine life, including the use of bird and bat deterrent systems and careful route planning for cable installation to avoid sensitive habitats. Regular environmental monitoring will be carried out to track the impact on marine ecosystems and adapt mitigation strategies as needed.
The decommissioning plan will include a comprehensive strategy for responsible removal of the turbines and cables at the end of the farm’s lifespan, minimizing any lasting environmental impact.
Hydropower Technologies
Hydropower, harnessing the energy of flowing water, represents a significant component of the global renewable energy portfolio. Its established technology and relatively high energy density make it a valuable tool in the fight against climate change, although its implementation necessitates careful consideration of environmental impacts. This section will explore different hydropower technologies and their associated environmental consequences, using specific examples to illustrate both successful and unsuccessful projects.Hydropower technologies can be broadly categorized into two main types: run-of-river and impoundment.
These categories differ significantly in their design, energy production capabilities, and environmental consequences. Understanding these differences is crucial for responsible hydropower development.
Run-of-River Hydropower
Run-of-river hydropower plants utilize the natural flow of a river to generate electricity. They typically involve diverting a portion of the river’s flow through a canal or pipeline to a turbine, after which the water is returned to the river. This approach minimizes the creation of large reservoirs, resulting in reduced land inundation and greenhouse gas emissions from decomposing organic matter.
However, run-of-river plants are subject to the variability of river flow, leading to fluctuating energy output. A successful example is the numerous small-scale run-of-river plants in Switzerland, which effectively integrate into the existing river systems while providing a substantial portion of the country’s renewable energy. Conversely, projects that fail to adequately consider the ecological needs of the river, such as those neglecting fish passage, can negatively impact aquatic ecosystems.
Impoundment Hydropower
Impoundment hydropower, in contrast, involves the construction of a dam to create a large reservoir. This reservoir stores water, which is then released to generate electricity through turbines. Impoundment projects can generate significantly more power than run-of-river systems, providing a consistent energy supply regardless of river flow variations. However, the creation of large reservoirs leads to significant environmental impacts, including habitat loss, altered river flow regimes, and increased greenhouse gas emissions from flooded vegetation.
The Three Gorges Dam in China, while a massive feat of engineering and a significant source of hydropower, exemplifies the trade-offs involved. Its construction displaced millions of people and altered the downstream ecosystem significantly. Conversely, poorly designed impoundment projects can suffer from issues like sedimentation, reducing reservoir capacity and lifespan.
Environmental Impact Comparison
Compared to other renewable energy sources, hydropower presents a mixed environmental profile. While it avoids direct greenhouse gas emissions during operation (unlike fossil fuels), the construction and operation of large impoundment projects can lead to significant habitat loss, methane emissions from decaying organic matter in reservoirs, and disruptions to river ecosystems. Solar and wind power, while requiring land use, generally have a smaller footprint and less significant ecosystem disruption.
However, hydropower offers a higher energy density and greater reliability compared to solar and wind, making it a crucial element in a diversified renewable energy mix. The environmental impact is highly dependent on the project’s design, location, and scale. Smaller, well-planned run-of-river projects often have a less severe environmental impact than large impoundment dams.
Examples of Hydropower Project Outcomes
The Itaipu Dam on the border of Brazil and Paraguay is frequently cited as a successful example of large-scale hydropower. Its substantial energy output has significantly contributed to both countries’ energy needs, while efforts have been made to mitigate environmental impacts through fish passage structures and ecological monitoring. Conversely, the Sardar Sarovar Dam in India, while providing significant irrigation benefits, faced strong opposition due to its displacement of local communities and its impact on downstream river ecosystems.
The differing outcomes highlight the importance of comprehensive environmental and social impact assessments before project initiation and the ongoing need for adaptive management strategies throughout the project lifecycle.
Geothermal Energy Technologies
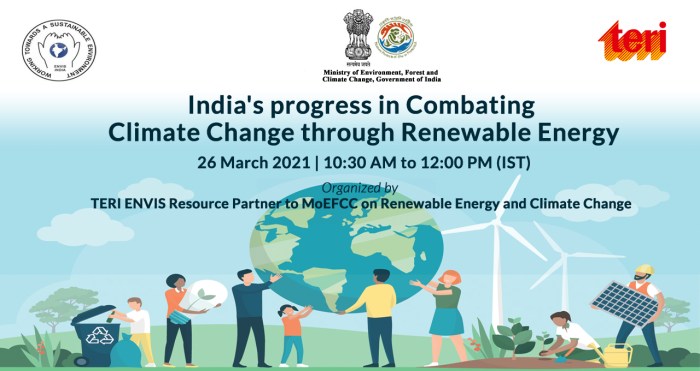
Source: teriin.org
Geothermal energy harnesses the heat stored within the Earth’s crust to generate electricity and provide direct heating. This renewable resource offers a consistent and reliable energy source, unlike solar or wind power which are intermittent. Exploiting this heat involves tapping into underground reservoirs of hot water or steam, a process that has evolved significantly over the years, leading to various power plant designs optimized for different geological conditions.Geothermal energy extraction relies on the principle of accessing naturally occurring geothermal reservoirs.
These reservoirs contain superheated water and steam under immense pressure. Wells are drilled deep into the Earth to reach these reservoirs, and the hot water or steam is then brought to the surface. The pressure difference between the reservoir and the surface causes the hot fluid to rise, and this energy is then used to drive turbines and generate electricity or directly used for heating purposes.
The specific methods employed vary depending on the characteristics of the geothermal resource.
Types of Geothermal Power Plants
Geothermal power plants are categorized based on the characteristics of the geothermal resource and the technology used to convert its heat energy into electricity. Three primary types exist: dry steam, flash steam, and binary cycle plants. Dry steam plants are the simplest, utilizing naturally occurring steam directly to drive turbines. Flash steam plants utilize hot water under high pressure, which flashes into steam as the pressure is reduced at the surface.
Binary cycle plants, on the other hand, employ a secondary working fluid with a lower boiling point than water, improving efficiency in lower temperature reservoirs.
Dry Steam Power Plants
Dry steam plants are the oldest and simplest type of geothermal power plant. They utilize naturally occurring steam directly from high-pressure geothermal reservoirs. This steam is piped directly to turbines, which rotate generators to produce electricity. The spent steam is then often condensed and reinjected into the reservoir to maintain pressure and sustainability. This method is only feasible in areas with naturally occurring high-pressure dry steam reservoirs, which are relatively rare.
An example of a dry steam plant is The Geysers geothermal field in California.
Flash Steam Power Plants
Flash steam plants are more common than dry steam plants because they can utilize hot water resources as well as steam. Hot water from the geothermal reservoir is brought to the surface under high pressure. As the pressure is reduced, a portion of the water flashes into steam. This steam then drives turbines to generate electricity, while the remaining hot water may be used for other purposes, such as direct heating, or reinjected into the reservoir.
This method is more adaptable to various geothermal resource types than dry steam plants.
Binary Cycle Power Plants
Binary cycle plants are particularly efficient in utilizing lower-temperature geothermal resources (around 100°C or more) which are more abundant than high-temperature resources. Instead of using the geothermal water directly to drive turbines, these plants use a secondary working fluid, such as isobutane or pentane, which has a lower boiling point. The heat from the geothermal water is transferred to the secondary fluid, causing it to vaporize and drive the turbine.
This method allows for greater efficiency in converting the geothermal heat energy into electricity, even with lower temperature resources.
Geothermal Energy vs. Fossil Fuels: Environmental Impact
A comparison of geothermal energy’s environmental impact versus fossil fuels reveals significant advantages for geothermal.
- Greenhouse Gas Emissions: Geothermal energy produces significantly fewer greenhouse gas emissions than fossil fuels. Fossil fuel combustion directly releases large quantities of CO2, while geothermal plants have minimal emissions, primarily consisting of small amounts of CO2 and other gases released from the geothermal fluids.
- Air Pollution: Geothermal power plants produce far less air pollution than fossil fuel plants. Fossil fuel combustion releases various pollutants such as sulfur oxides, nitrogen oxides, and particulate matter, which contribute to respiratory problems and acid rain. Geothermal plants produce negligible amounts of these pollutants.
- Water Usage: While geothermal plants do use water, the water usage is generally lower than many fossil fuel power plants, particularly those using cooling towers. Furthermore, the water is often reinjected back into the reservoir, minimizing environmental impact. Fossil fuel power plants require large quantities of water for cooling purposes.
- Land Use: The land footprint of geothermal power plants is relatively small compared to fossil fuel power plants, especially considering the vast areas required for coal mining or oil and gas extraction.
- Waste Disposal: Geothermal plants produce minimal waste, primarily consisting of the treated geothermal fluids that are often reinjected into the reservoir. Fossil fuel power plants generate large amounts of waste, including ash, slag, and spent fuel, requiring costly and environmentally challenging disposal methods.
Biomass Energy Technologies
Biomass energy harnesses the chemical energy stored in organic matter, offering a renewable energy source. This energy can be converted into various usable forms, addressing the global need for sustainable energy alternatives. Different technologies exist for transforming biomass into usable energy, each with its own advantages and drawbacks.Biomass energy generation methods encompass a range of approaches, each utilizing different aspects of the biomass material.
The choice of method often depends on factors such as the type of biomass available, the desired energy output, and the overall cost-effectiveness. Sustainability concerns, however, are paramount and must be carefully considered in any biomass energy project.
Methods of Biomass Energy Generation
Biomass energy can be harnessed through several primary methods: biofuels, biogas, and direct biomass combustion. Biofuels involve converting biomass into liquid or gaseous fuels suitable for transportation or other applications. Biogas production utilizes anaerobic digestion to break down organic matter, generating a mixture of methane and carbon dioxide. Direct biomass combustion involves burning biomass directly to generate heat or electricity.
Each method presents unique advantages and challenges regarding efficiency, environmental impact, and economic viability.
Biofuel Production
Biofuels are liquid or gaseous fuels derived from biomass. Examples include ethanol, produced through fermentation of sugars from crops like corn or sugarcane, and biodiesel, created through transesterification of vegetable oils or animal fats. These biofuels can be used as substitutes or blends with conventional fossil fuels in transportation sectors. The production process, however, can be resource-intensive and may compete with food production if not managed sustainably.
Biogas Production
Biogas is a mixture of methane (CH₄) and carbon dioxide (CO₂) produced through the anaerobic digestion of organic matter. This process involves breaking down organic waste in the absence of oxygen by microorganisms. Biogas can be used directly as a fuel source for heating or cooking, or it can be upgraded to biomethane, a renewable natural gas substitute. Anaerobic digesters, the facilities where this process takes place, can be designed for various scales, from small-scale farm digesters to large-scale municipal waste treatment plants.
Biomass Combustion
Direct biomass combustion involves burning biomass directly to generate heat or electricity. This method is commonly employed in power plants, where large quantities of biomass are burned to produce steam, which drives turbines to generate electricity. Smaller-scale applications include using wood stoves or biomass boilers for heating homes and businesses. While relatively simple and efficient in energy conversion, direct combustion can contribute to air pollution if not properly managed, requiring the use of emission control technologies.
Sustainability Concerns in Biomass Energy Production
While biomass is renewable, its unsustainable production can lead to several environmental problems. Deforestation for biomass plantations can lead to habitat loss and biodiversity reduction. The intensive use of fertilizers and pesticides in biomass crop production can pollute water sources and harm ecosystems. Furthermore, the transportation and processing of biomass can contribute to greenhouse gas emissions. Careful consideration of these factors is crucial to ensure the long-term sustainability of biomass energy.
Sustainable Biomass Energy Practices
Sustainable biomass energy practices focus on minimizing negative environmental impacts while maximizing energy production. This includes using agricultural residues and waste materials as feedstock, reducing reliance on food crops for biofuel production. Employing sustainable forestry practices, such as selective logging and reforestation, helps ensure a continuous supply of biomass without deforestation. Furthermore, optimizing energy conversion efficiency through advanced technologies and implementing strict emission control measures minimizes the environmental footprint of biomass energy.
Examples include using dedicated energy crops grown on marginal lands, improving anaerobic digestion processes for higher biogas yields, and implementing carbon capture and storage technologies in biomass power plants.
Energy Storage Technologies
The intermittent nature of renewable energy sources like solar and wind power presents a significant challenge to their widespread adoption. The sun doesn’t always shine, and the wind doesn’t always blow, creating fluctuations in energy supply. This is where energy storage technologies become crucial, acting as a buffer to ensure a consistent and reliable energy flow, maximizing the utilization of renewable energy and improving grid stability.
Efficient and cost-effective energy storage is essential for a truly sustainable energy future.Energy storage technologies bridge the gap between energy generation and consumption, smoothing out the variability inherent in renewable sources. Different technologies offer varying levels of efficiency, scalability, and cost-effectiveness, each with its own set of advantages and disadvantages. Choosing the right technology depends on factors such as the scale of the application (residential, grid-scale), the duration of storage required, and the overall cost considerations.
Types of Energy Storage Technologies
Several technologies are currently employed or under development for energy storage. These include batteries, pumped hydro storage, and compressed air energy storage (CAES), each offering unique characteristics and applications. Batteries are widely used for smaller-scale applications, while pumped hydro and CAES are better suited for larger-scale grid-level storage.
Comparison of Energy Storage Technologies, Green energy technologies for combating climate change
Batteries, pumped hydro storage, and compressed air energy storage represent three distinct approaches to energy storage, each with its own strengths and weaknesses. Batteries, particularly lithium-ion batteries, are versatile and scalable, suitable for various applications from electric vehicles to grid-scale storage. Pumped hydro storage leverages existing hydropower infrastructure or geographically suitable locations to store energy as potential energy in water reservoirs at different elevations.
Compressed air energy storage utilizes the compression and expansion of air to store and release energy.
Energy Storage Technology | Advantages | Disadvantages | Typical Applications |
---|---|---|---|
Batteries (Lithium-ion) | High energy density, fast response times, modular and scalable, relatively low environmental impact (compared to fossil fuels). | Limited lifespan, relatively high cost per kWh, potential safety concerns, requires rare earth minerals for manufacturing. | Electric vehicles, residential energy storage, grid-scale storage (increasingly common). |
Pumped Hydro Storage | Long duration storage, mature technology, relatively low cost per kWh (once infrastructure is in place), high efficiency. | Geographically limited, large land footprint, significant initial capital investment, environmental impact (on surrounding ecosystems). | Grid-scale energy storage, peak demand management. |
Compressed Air Energy Storage (CAES) | Long duration storage potential, can utilize existing infrastructure (e.g., depleted gas fields), relatively low environmental impact. | Lower round-trip efficiency compared to pumped hydro, high initial capital cost, potential for noise pollution. | Grid-scale energy storage, particularly in areas with suitable geological formations. |
Smart Grid Technologies
Smart grids represent a critical advancement in electricity distribution, particularly vital for effectively integrating the intermittent nature of renewable energy sources into the power system. They offer a sophisticated approach to managing electricity flow, enhancing reliability, and improving overall efficiency. This intelligent infrastructure allows for a more dynamic and responsive energy network, better accommodating the fluctuating output of solar and wind power.Smart grids optimize renewable energy distribution by providing a platform for real-time monitoring and control of energy generation, transmission, and consumption.
This allows for better forecasting of energy supply and demand, enabling grid operators to balance fluctuating renewable energy sources with traditional power plants and efficiently manage energy storage resources. The system’s intelligence facilitates the integration of distributed generation, such as rooftop solar panels, into the broader energy grid, maximizing the utilization of locally produced renewable energy.
Technological Components of a Smart Grid
A smart grid relies on a complex interplay of advanced technologies to achieve its objectives. These technologies work together to create a highly responsive and efficient energy network. The core components include sophisticated sensors, advanced communication networks, and intelligent control systems.
- Sensors: These devices are strategically placed throughout the grid to monitor various parameters, such as voltage, current, frequency, and power flow. Data collected by these sensors provides real-time information about the grid’s condition, enabling proactive management and rapid response to disruptions. Examples include smart meters installed in homes and businesses, providing granular data on energy consumption, and sensors on transmission lines that monitor temperature and current load.
- Communication Networks: Robust and reliable communication networks are essential for transmitting the vast amounts of data collected by sensors to control centers and other grid components. These networks utilize various technologies, including fiber optics, wireless communication (such as cellular and Wi-Fi), and power line communication (PLC), to ensure seamless data flow across the grid. The choice of technology often depends on the specific needs of the location and the type of data being transmitted.
- Control Systems: Advanced control systems utilize the data collected by sensors and transmitted via communication networks to manage the flow of electricity across the grid. These systems employ sophisticated algorithms and artificial intelligence to optimize energy distribution, predict potential problems, and respond to unexpected events such as power outages or surges in demand. This allows for improved grid stability and enhanced reliability.
Challenges and Benefits of Implementing Smart Grid Technologies
Implementing smart grid technologies presents several challenges, but the potential benefits significantly outweigh the difficulties.
- Challenges: High initial investment costs, the need for robust cybersecurity measures to protect against cyberattacks, and the complexity of integrating various technologies and systems across a vast geographical area are significant hurdles. Furthermore, the standardization of communication protocols and data formats remains a challenge, hindering seamless interoperability between different grid components from various vendors.
- Benefits: Improved grid reliability and resilience, reduced energy losses during transmission and distribution, increased integration of renewable energy sources, better energy efficiency, and enhanced grid flexibility to accommodate fluctuating energy demands are some key advantages. Furthermore, smart grids enable better demand-side management, empowering consumers to actively participate in optimizing energy usage and reducing their carbon footprint. For example, the smart grid in California has demonstrated significant improvements in grid stability and integration of renewable energy, reducing reliance on fossil fuel-based power plants.
Policy and Economic Aspects of Green Energy Transition
The shift towards a green energy economy is not merely an environmental imperative; it’s a complex interplay of policy decisions and economic realities. Government intervention plays a crucial role in shaping the speed and direction of this transition, while the economic implications are far-reaching, impacting both opportunities and challenges.Government policies significantly influence the adoption of green energy technologies. Incentives, regulations, and investments all contribute to creating a favorable environment for renewable energy development.
Without supportive policies, the transition would be significantly slower and less efficient, hindered by the inherent higher upfront costs associated with many green energy technologies compared to fossil fuels.
Government Policies Promoting Green Energy Adoption
Effective government policies are essential for driving the widespread adoption of green energy. These policies can take many forms, including financial incentives like tax credits and subsidies for renewable energy projects, feed-in tariffs guaranteeing a minimum price for renewable energy produced, and mandates requiring a certain percentage of electricity to come from renewable sources. Furthermore, policies can streamline permitting processes for renewable energy infrastructure and invest in research and development of new technologies.
Stronger environmental regulations that put a price on carbon emissions or limit the use of fossil fuels also create a more competitive landscape for green energy. Conversely, a lack of supportive policies, or even policies actively hindering renewable energy development, can severely impede progress.
Economic Benefits of the Green Energy Transition
The transition to a green energy economy offers substantial economic benefits. The creation of new jobs in manufacturing, installation, maintenance, and research of renewable energy technologies is a major driver of economic growth. Furthermore, reduced reliance on imported fossil fuels enhances energy security and reduces vulnerability to global price fluctuations. Green energy investments also stimulate innovation and technological advancements, leading to long-term economic competitiveness.
The health benefits resulting from reduced air pollution contribute to increased productivity and reduced healthcare costs. Finally, attracting green investments can boost a nation’s image and competitiveness on the global stage.
Economic Challenges of the Green Energy Transition
Despite the considerable economic benefits, the transition to a green energy economy also presents challenges. The initial high capital costs of renewable energy infrastructure can be a significant barrier to entry, especially for smaller businesses and developing countries. Intermittency of renewable energy sources like solar and wind necessitates the development and deployment of efficient energy storage solutions, adding further cost and complexity.
The need for workforce retraining and upskilling to adapt to the changing energy landscape also presents a significant challenge. Moreover, managing the potential job losses in the fossil fuel industry requires careful planning and proactive measures to ensure a just transition for affected workers.
Examples of Successful and Unsuccessal Government Initiatives
Germany’s Energiewende policy, aiming for a rapid transition to renewable energy, has seen considerable success in increasing renewable energy capacity. However, it has also faced challenges related to grid stability and cost management. Denmark’s long-standing support for wind energy has led to it becoming a global leader in wind power, demonstrating the effectiveness of consistent and long-term policy support.
In contrast, some countries have seen their renewable energy targets fall short due to insufficient policy support, inconsistent regulatory frameworks, or a lack of investment in grid infrastructure. The failure to adequately address the social and economic implications of the transition, such as job losses in traditional energy sectors, can also lead to resistance and hinder progress.
Future Trends in Green Energy Technologies
The rapid advancement of green energy technologies is crucial for mitigating climate change and achieving global net-zero emissions targets. Ongoing research and development are constantly pushing the boundaries of what’s possible, leading to more efficient, cost-effective, and sustainable energy solutions. This section explores some of the most promising emerging trends shaping the future of green energy.Emerging trends in green energy research and development are characterized by a focus on increased efficiency, reduced costs, and improved integration with existing energy infrastructure.
This involves exploring novel materials, optimizing energy conversion processes, and developing smarter grid management systems. Significant progress is being made across various sectors, driving the transition towards a cleaner energy future.
Innovative Technologies: Wave and Tidal Energy
Harnessing the immense power of ocean waves and tides presents a significant opportunity for sustainable energy generation. Wave energy converters utilize the kinetic energy of ocean waves to generate electricity through various mechanisms, such as oscillating water columns or point absorbers. Tidal energy, on the other hand, leverages the predictable rise and fall of tides to drive turbines, similar to hydropower systems.
While still in their relatively early stages of development, both technologies hold immense potential, particularly in coastal regions with strong wave action or significant tidal ranges. For example, the European Marine Energy Centre (EMEC) in Orkney, Scotland, serves as a testing ground for various wave and tidal energy technologies, demonstrating their feasibility and providing valuable data for future development.
The consistent and predictable nature of these energy sources, unlike solar and wind, offers a valuable complement to the intermittent nature of other renewables.
Technological Advancements and Net-Zero Emissions
Technological advancements play a pivotal role in achieving net-zero emissions targets. Improvements in solar panel efficiency, for example, are continuously reducing the land area required for large-scale solar farms. Similarly, advancements in wind turbine design are leading to larger and more efficient turbines, capable of capturing more wind energy. Beyond individual technologies, the development of smart grids is crucial for effectively managing the intermittent nature of renewable energy sources.
Smart grids utilize advanced sensors, data analytics, and communication technologies to optimize energy distribution, integrate renewable energy sources seamlessly, and reduce energy waste. Furthermore, breakthroughs in energy storage technologies, such as advanced battery systems and pumped hydro storage, are crucial for ensuring grid stability and reliability when renewable energy sources are unavailable. The integration of these advancements, coupled with policy support and public awareness, will be essential in achieving a sustainable and carbon-neutral energy future.
The ambitious goal of net-zero emissions by 2050, as adopted by many countries, relies heavily on these continued technological breakthroughs and their successful implementation. The success of this transition will depend on collaboration between researchers, policymakers, and industry stakeholders to overcome the remaining technological and economic challenges.
Closing Summary: Green Energy Technologies For Combating Climate Change
Ultimately, combating climate change requires a multifaceted approach that leverages the full potential of green energy technologies. While challenges remain – including infrastructure development, technological limitations, and policy hurdles – the advancements in renewable energy sources and related technologies offer a beacon of hope. A concerted global effort, integrating technological innovation with effective policy frameworks and public awareness, is essential to successfully navigate this transition and secure a sustainable future for generations to come.
The path forward demands collaboration, investment, and a collective commitment to building a cleaner, greener world.
FAQ Insights
What are the main environmental drawbacks of solar energy?
Manufacturing solar panels requires energy and resources, and some materials can be toxic. End-of-life disposal of panels is also a growing concern requiring responsible recycling solutions. Land use for large-scale solar farms can impact ecosystems, although this is often less impactful than fossil fuel extraction.
How does wind energy affect wildlife?
Wind turbines can pose a risk to birds and bats, particularly through collisions. Careful site selection, turbine design modifications, and operational adjustments are implemented to minimize these impacts. Further research and technological improvements continue to address these concerns.
What are the limitations of hydropower?
Hydropower projects can significantly alter river ecosystems, impacting aquatic life and water flow. Large dams can also displace communities and contribute to greenhouse gas emissions from decaying organic matter in reservoirs. The availability of suitable sites for hydropower is geographically limited.
How effective are current energy storage technologies?
Current energy storage solutions are improving rapidly, but limitations remain in terms of cost, scalability, and energy density. While batteries are becoming more efficient and affordable, other technologies like pumped hydro and compressed air energy storage offer advantages in specific applications, but also have their own limitations.
What is the role of smart grids in the energy transition?
Smart grids enhance the integration of renewable energy sources by optimizing energy distribution, improving grid stability, and reducing energy waste. They achieve this through advanced sensors, communication networks, and control systems that allow for real-time monitoring and management of energy flow.