Innovative Renewable Energy Technologies A Comprehensive Overview
Innovative renewable energy technologies are rapidly transforming the global energy landscape. This exploration delves into the latest advancements in solar, wind, wave, tidal, and geothermal energy, examining their efficiency, cost-effectiveness, and environmental impact. We will also investigate innovative energy storage solutions, crucial for addressing the intermittency of renewable sources, and explore the role of smart grids in optimizing renewable energy integration.
Finally, we will analyze the policy, economic, and environmental considerations driving the transition to a sustainable energy future.
The shift towards renewable energy is not merely an environmental imperative; it presents significant economic opportunities and technological advancements. This comprehensive overview aims to provide a balanced perspective, highlighting both the potential benefits and challenges associated with each technology and approach. From cutting-edge battery technologies to sophisticated grid management systems, we will examine the key drivers shaping the future of renewable energy.
Emerging Trends in Renewable Energy: Innovative Renewable Energy Technologies
The global shift towards sustainable energy sources is accelerating, driven by climate change concerns and technological advancements. This section explores key emerging trends in renewable energy technologies, focusing on recent progress and future potential. We will examine advancements in solar, wind, wave/tidal, and geothermal energy, considering efficiency improvements, cost reductions, and environmental implications.
Solar Energy Advancements
Recent years have witnessed significant strides in solar energy technology, primarily focused on increasing efficiency and reducing production costs. Perovskite solar cells, for example, are emerging as a promising alternative to traditional silicon-based cells, offering potentially higher efficiencies and lower manufacturing costs. Improvements in silicon cell manufacturing techniques, such as advancements in wafering and passivation, have also led to notable efficiency gains.
Furthermore, the integration of solar panels into building materials (Building Integrated Photovoltaics or BIPV) is gaining traction, reducing land use and improving aesthetics. Cost reductions are being driven by economies of scale, automation in manufacturing, and government subsidies. For instance, the cost of solar photovoltaic (PV) modules has decreased dramatically over the past decade, making solar energy increasingly competitive with fossil fuels in many regions.
Next-Generation Wind Turbine Designs, Innovative renewable energy technologies
The pursuit of higher energy capture in wind energy is leading to the development of larger and more efficient wind turbines. This includes the design of longer blades, utilizing advanced materials like carbon fiber composites to withstand higher wind speeds and reduce weight. Floating offshore wind turbines are also gaining prominence, allowing for the exploitation of higher wind speeds in deeper waters.
However, challenges remain, including the high initial investment costs associated with these larger and more complex structures, the logistical complexities of installation and maintenance in offshore environments, and the potential impact on marine ecosystems. Research is ongoing to optimize blade design, improve energy conversion efficiency, and reduce the environmental footprint of these large-scale projects. For example, advancements in direct-drive generators are eliminating the need for gearboxes, enhancing reliability and reducing maintenance needs.
Wave and Tidal Energy Potential
Wave and tidal energy represent untapped renewable resources with significant potential. These technologies harness the kinetic energy of ocean currents and waves to generate electricity. Wave energy converters (WECs) come in various designs, from oscillating water columns to point absorbers, each with its own advantages and disadvantages concerning energy capture efficiency and survivability in harsh marine conditions. Tidal energy, on the other hand, typically utilizes tidal barrages or turbines placed in tidal currents.
Advantages include the predictability of tidal cycles and high energy density in suitable locations. However, limitations include high initial capital costs, environmental impacts on marine ecosystems (e.g., disruption of fish migration patterns), and the geographic constraints of suitable sites. Currently, wave and tidal energy are still in their early stages of development, with limited commercial deployment.
Geothermal Energy Extraction Methods
Geothermal energy harnesses the heat from the Earth’s interior. Different methods exist for extracting this energy, each with its own environmental implications. Enhanced Geothermal Systems (EGS) involve drilling deep into the Earth’s crust to create artificial geothermal reservoirs, potentially accessing vast geothermal resources. However, concerns exist regarding induced seismicity and water usage. Traditional hydrothermal systems utilize naturally occurring geothermal reservoirs, which are generally less impactful environmentally but are geographically limited.
Geopressured systems exploit the energy from hot, pressurized water found in deep sedimentary formations. The environmental impact of geothermal energy extraction varies depending on the method employed, with potential impacts including greenhouse gas emissions (from some systems) and water contamination. Careful site selection, responsible resource management, and the development of advanced technologies are crucial for minimizing environmental impacts.
Technology | Efficiency | Cost | Environmental Impact |
---|---|---|---|
Solar PV (Silicon) | Up to 25% (increasing) | Decreasing significantly | Low (manufacturing impacts, land use) |
Solar PV (Perovskite) | Potential for >30% | Potentially lower than silicon | Research ongoing on long-term environmental impacts |
Wind Turbines (Onshore) | ~40-50% | Moderate | Visual impact, bird and bat mortality |
Wind Turbines (Offshore) | ~50-60% (potential) | High (initial investment) | Marine ecosystem impacts (potential) |
Wave Energy | Variable, research ongoing | High (currently) | Marine ecosystem impacts (potential) |
Tidal Energy | Variable, depending on technology | High (currently) | Marine ecosystem impacts (potential) |
Geothermal (Hydrothermal) | Variable, depending on resource | Moderate to High | Relatively low (compared to other methods, potential for induced seismicity) |
Geothermal (EGS) | Potential for high output | High (currently) | Potential for induced seismicity, water usage |
Innovative Energy Storage Solutions
The intermittent nature of renewable energy sources like solar and wind necessitates efficient and reliable energy storage solutions. These technologies are crucial for ensuring a consistent power supply, enhancing grid stability, and maximizing the utilization of renewable energy resources. This section will explore several key energy storage technologies, their functionalities, and their respective advantages and disadvantages.
Lithium-ion Battery Technology
Lithium-ion batteries are currently the dominant technology in the renewable energy storage market. Their widespread adoption is due to their high energy density, relatively long lifespan, and relatively quick charging times. These batteries operate through the intercalation of lithium ions between the cathode and anode materials during charging and discharging. The cathode, typically a layered metal oxide, accepts lithium ions, while the anode, often graphite, releases them.
This movement of ions generates an electric current. Different chemistries exist, such as lithium iron phosphate (LFP) and nickel manganese cobalt (NMC), each with varying performance characteristics and cost implications. LFP batteries are known for their safety and longer lifespan, while NMC batteries offer higher energy density but may pose slightly greater safety concerns.
Flow Battery Technology
Flow batteries differ significantly from lithium-ion batteries in their operational principle. Instead of storing energy within the electrodes, flow batteries store energy in liquid electrolytes that are pumped through an electrochemical cell. The amount of energy stored is directly proportional to the volume of the electrolyte. This feature allows for independent scaling of power and energy capacity, offering flexibility in design and application.
Innovative renewable energy technologies are rapidly advancing, offering promising solutions to combat climate change. To stay abreast of the leading developments, it’s beneficial to keep an eye on the key players; for example, you can check out this list of Top green energy companies to watch in 2025 for insights into the future of the sector. This will help you understand which companies are driving the most impactful innovations in renewable energy sources.
Vanadium redox flow batteries (VRFBs) are a prominent example, utilizing vanadium ions in different oxidation states to store and release energy. Flow batteries are particularly well-suited for long-duration energy storage applications, but their higher cost and lower energy density compared to lithium-ion batteries currently limit their widespread adoption.
Solid-State Battery Technology
Solid-state batteries represent a promising advancement in energy storage, replacing the liquid or gel electrolytes in conventional lithium-ion batteries with solid electrolytes. This change offers several potential advantages, including enhanced safety (reduced flammability), higher energy density, and potentially longer lifespan. However, challenges remain in developing solid electrolytes with high ionic conductivity and scalability for mass production. Solid-state batteries are still under intensive research and development, and their widespread commercialization is anticipated in the coming years.
Pumped Hydro Storage and Compressed Air Energy Storage
Pumped hydro storage (PHS) utilizes the potential energy of water stored at a higher elevation. During periods of excess renewable energy generation, water is pumped uphill to a reservoir. When energy is needed, the water flows downhill, driving turbines to generate electricity. PHS systems are well-established, offering long lifespans and high efficiency, but they require significant geographical constraints and large water resources.
Compressed air energy storage (CAES) involves compressing air during periods of low energy demand and storing it in underground caverns or depleted gas fields. When energy is required, the compressed air is released to drive turbines. CAES offers a potentially large-scale storage solution, but its efficiency is somewhat lower than PHS due to energy losses during compression and expansion.
Innovative Thermal Energy Storage Systems
Thermal energy storage (TES) systems store energy in the form of heat or cold. Sensible heat storage involves storing energy by changing the temperature of a material, such as water, molten salts, or rocks. Latent heat storage utilizes the energy absorbed or released during phase transitions, such as melting or freezing. For example, phase-change materials (PCMs) can store large amounts of energy with relatively small temperature changes.
TES systems find applications in various sectors, including solar thermal power plants, district heating and cooling networks, and industrial processes. However, limitations include heat losses during storage and the need for efficient heat transfer mechanisms.
Comparison of Energy Storage Technologies
The choice of energy storage technology depends heavily on the specific application and its requirements. The following table summarizes the key advantages and disadvantages of the technologies discussed:
Technology | Cost | Lifespan | Environmental Impact |
---|---|---|---|
Lithium-ion | Moderate to High (decreasing) | Moderate (5-10 years) | Moderate (material sourcing and recycling concerns) |
Flow Batteries | High | Long (15-20+ years) | Low to Moderate (depending on electrolyte chemistry) |
Solid-State Batteries | High (currently) | Potentially Very Long | Potentially Low (depending on material choices) |
Pumped Hydro | High (initial investment) | Very Long (50+ years) | Low |
Compressed Air | Moderate to High | Moderate to Long | Low to Moderate (depending on site selection and construction) |
Thermal Energy Storage | Moderate to High (depending on technology) | Long (depending on material and design) | Low to Moderate (depending on material choices) |
Smart Grid Integration of Renewable Energy and Storage
A conceptual diagram illustrating the integration of renewable energy sources (solar, wind) and various energy storage systems (lithium-ion batteries, pumped hydro) within a smart grid would show renewable energy generators connected to the grid via inverters. The smart grid would monitor energy production and consumption in real-time, utilizing advanced algorithms to optimize energy dispatch and storage management. Excess renewable energy would be directed to energy storage systems, while stored energy would be released to the grid during periods of peak demand or low renewable generation.
The system would include communication networks for data exchange and control, allowing for dynamic adjustment based on real-time conditions. This integrated system enhances grid stability, reliability, and the overall utilization of renewable energy resources. The system could also incorporate demand-side management strategies to further optimize energy usage.
Smart Grid Integration and Management
The integration of renewable energy sources, such as solar and wind power, into existing power grids presents both significant opportunities and considerable challenges. Smart grids, with their advanced communication and control systems, are crucial for effectively managing the intermittent nature of renewable energy and ensuring grid stability and reliability. This section explores key aspects of smart grid integration and management in the context of renewable energy.Successful Smart Grid Implementations Leveraging Renewable EnergySeveral regions have successfully integrated renewable energy into their smart grids.
Denmark, for example, has made significant strides in integrating wind power, utilizing advanced forecasting techniques and grid management strategies to accommodate its variable output. Similarly, Germany’s Energiewende program, while facing challenges, showcases large-scale integration of solar and wind power through smart grid technologies. These implementations often involve sophisticated energy management systems that optimize energy distribution based on real-time data and predictions.
The success of these initiatives hinges on robust communication infrastructure, advanced metering infrastructure (AMI), and effective demand-side management programs.Key Challenges in Integrating Intermittent Renewable Energy SourcesThe intermittent nature of renewable energy sources like solar and wind poses significant challenges to grid stability. The unpredictable fluctuations in power generation can lead to frequency variations and voltage instability, requiring sophisticated control mechanisms to maintain grid equilibrium.
Furthermore, integrating large amounts of distributed generation from renewable sources necessitates upgrades to existing grid infrastructure, including transmission lines and substations, to handle the increased capacity and accommodate the bidirectional flow of power. Balancing supply and demand in real-time becomes significantly more complex with the introduction of unpredictable renewable energy sources. Accurate forecasting and flexible grid operations are vital for mitigating these challenges.Advanced Sensors and Data Analytics for Optimizing Renewable Energy Grid IntegrationAdvanced sensors play a critical role in monitoring and managing renewable energy integration.
These sensors collect real-time data on power generation, grid conditions, and energy consumption. Data analytics techniques, including time series analysis and machine learning algorithms, process this data to identify patterns, predict future energy production, and optimize grid operations. For instance, sensors on wind turbines provide data on wind speed and power output, allowing for more accurate forecasting and scheduling of energy dispatch.
Smart meters provide granular data on energy consumption patterns, enabling demand-side management strategies to better match supply and demand.Artificial Intelligence and Machine Learning for Forecasting and Management of Renewable Energy GenerationArtificial intelligence (AI) and machine learning (ML) algorithms are increasingly used to improve the forecasting and management of renewable energy generation. These algorithms can analyze vast amounts of historical data, weather forecasts, and other relevant information to predict future energy output with greater accuracy than traditional methods.
This improved forecasting allows grid operators to better manage the intermittent nature of renewable energy, reducing the need for expensive backup power sources and enhancing grid stability. Furthermore, AI and ML can optimize the operation of energy storage systems, ensuring that energy is stored and discharged effectively to balance supply and demand.Data Flow and Decision-Making Processes within a Smart Grid System Utilizing Renewable EnergyThe following flowchart illustrates a simplified representation of data flow and decision-making within a smart grid system utilizing renewable energy:[A textual representation of a flowchart is provided below, since image creation is outside the scope of this response.
The actual flowchart would be visual.] Flowchart:
1. Data Acquisition
Innovative renewable energy technologies are rapidly evolving, offering exciting possibilities for a sustainable future. A key aspect of this progress involves empowering local communities, which is why initiatives like Community-driven renewable energy projects are so important. These projects not only increase access to clean energy but also foster local ownership and expertise in these cutting-edge technologies, further accelerating innovation in the field.
Sensors on renewable energy sources (solar panels, wind turbines), smart meters, and grid infrastructure collect real-time data on generation, consumption, and grid conditions.
2. Data Transmission
Data is transmitted to a central control system via communication networks.
3. Data Processing
Data is processed using AI/ML algorithms for forecasting, anomaly detection, and optimization.
Innovative renewable energy technologies are crucial for a sustainable future, offering diverse solutions for various contexts. A prime example showcasing the positive impact of such technologies is wind power, particularly its advantages for underserved areas. For a detailed look at how this plays out, explore the significant benefits of wind power for rural communities by visiting this informative resource: Benefits of wind power for rural communities.
Ultimately, continued investment in innovative renewable energy technologies is vital for global progress.
4. Prediction and Optimization
Forecasts of renewable energy generation are made, and optimal strategies for energy dispatch, storage, and demand-side management are determined.
5. Grid Control
Control signals are sent to various grid components (generators, storage systems, demand-response programs) to adjust operations based on the optimization strategies.
6. Feedback and Monitoring
The system continuously monitors grid performance and adjusts control actions based on real-time feedback.
Policy and Economic Aspects of Renewable Energy
The transition to a renewable energy-based economy hinges on a complex interplay of government policies, economic incentives, and technological advancements. Understanding the economic feasibility and potential barriers to widespread adoption is crucial for effective planning and implementation. This section explores the key policy and economic drivers shaping the renewable energy landscape.
Government Policies and Subsidies
Government policies play a pivotal role in accelerating the development and deployment of renewable energy technologies. Subsidies, tax credits, feed-in tariffs, and renewable portfolio standards (RPS) significantly influence investment decisions and market penetration. For example, the substantial tax credits offered in the United States under the Inflation Reduction Act have spurred significant investment in solar and wind energy projects.
Conversely, the absence or phasing out of such incentives can hinder growth, as seen in some European countries where support for solar has decreased. Effective policy design requires a balance between providing sufficient incentives to stimulate innovation and ensuring long-term market stability. This often involves carefully considering the lifecycle costs of renewable energy projects, factoring in potential fluctuations in energy prices and technological advancements.
Economic Feasibility of Renewable Energy Projects
The economic feasibility of renewable energy projects is assessed through a comprehensive analysis of capital costs, operating costs, and energy prices. Capital costs encompass the initial investment in equipment, infrastructure, and land acquisition. Operating costs include maintenance, repair, and insurance. Energy prices, both current and projected, determine the revenue stream generated by the project. A detailed financial model, incorporating these factors, is essential for evaluating the return on investment (ROI) and determining the project’s viability.
For instance, a large-scale solar farm might have high initial capital costs but low operating costs, resulting in a competitive ROI over its lifespan, especially in regions with high solar irradiance and favorable electricity prices. Conversely, a smaller-scale project may face higher per-unit costs and require careful financial planning to ensure profitability.
Barriers to Widespread Adoption and Mitigation Strategies
Several barriers impede the widespread adoption of renewable energy technologies. These include high upfront capital costs, intermittency of renewable energy sources (like solar and wind), grid integration challenges, and regulatory hurdles. Strategies to overcome these barriers include innovative financing mechanisms, such as green bonds and crowdfunding, advancements in energy storage technologies (e.g., battery storage, pumped hydro), smart grid infrastructure development to better manage fluctuating energy supply, and streamlined permitting processes.
Public awareness campaigns can also play a vital role in overcoming public perception challenges and fostering wider acceptance of renewable energy solutions.
Economic Benefits and Social Impacts of a Renewable Energy Transition
The transition to a renewable energy-based economy offers significant economic and social benefits. Economic benefits include job creation in the manufacturing, installation, and maintenance sectors, reduced dependence on fossil fuels and volatile global energy markets, and increased energy security. Social benefits encompass improved air and water quality, reduced greenhouse gas emissions, and enhanced public health. However, the transition also presents challenges, such as potential job displacement in fossil fuel industries, the need for workforce retraining, and the equitable distribution of benefits and costs across different communities.
Careful planning and policy implementation are crucial to ensure a just and equitable transition that maximizes benefits while minimizing negative impacts.
Key Economic Indicators and Policy Recommendations
Economic Indicator | Current Status/Trend | Policy Recommendation |
---|---|---|
Levelized Cost of Energy (LCOE) for renewables | Decreasing for solar and wind, but varies by location and technology | Continue supporting R&D to further reduce LCOE; incentivize deployment in favorable locations. |
Investment in renewable energy infrastructure | Increasing globally, but uneven distribution across regions | Establish clear and stable long-term policy frameworks; improve access to finance for renewable energy projects. |
Carbon emissions from the energy sector | Needs significant reduction to meet climate goals | Implement carbon pricing mechanisms; strengthen renewable portfolio standards (RPS). |
Environmental Impact and Sustainability
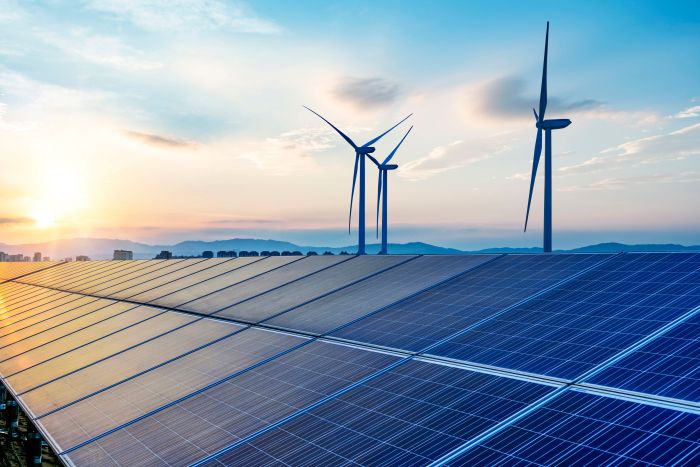
Source: com.au
The transition to renewable energy sources presents a crucial opportunity to mitigate climate change and improve environmental quality. While renewable energy technologies offer significant environmental advantages over fossil fuels, it’s essential to understand their potential impacts and develop strategies for sustainable implementation. This section will explore the environmental benefits and drawbacks of various renewable energy technologies, outlining methods for minimizing their footprint and promoting environmentally responsible practices.The environmental benefits of transitioning to renewable energy sources are substantial.
Unlike fossil fuels, which release greenhouse gases (GHGs) contributing to global warming and air pollution, renewable energy sources like solar, wind, hydro, and geothermal produce little to no GHG emissions during operation. This drastically reduces the contribution to climate change, improving air quality and public health. The reduced reliance on fossil fuels also minimizes habitat destruction associated with extraction and transportation.
Environmental Impacts of Renewable Energy Technologies
Different renewable energy technologies have varying environmental impacts. For instance, large-scale hydropower projects can alter river ecosystems and displace communities. Solar and wind farms require significant land areas, potentially impacting habitats and landscapes. The manufacturing and disposal of renewable energy technologies also generate waste, although typically less than fossil fuel counterparts. Water consumption varies significantly; some technologies, like concentrated solar power, have higher water demands than others, like wind power.
Strategies for Minimizing Environmental Footprint
Minimizing the environmental impact of renewable energy projects requires a multi-faceted approach. Careful site selection is crucial, prioritizing areas with minimal ecological sensitivity and avoiding environmentally sensitive habitats. Employing sustainable land management practices, such as reforestation and habitat restoration, can mitigate land use impacts. Water-efficient technologies and closed-loop water systems can reduce water consumption. Furthermore, designing for efficient resource use during manufacturing, extending the operational lifespan of technologies, and developing effective recycling and waste management strategies are essential for reducing the overall environmental footprint.
Innovative renewable energy technologies, such as solar and wind power, are not only environmentally beneficial but also significant economic drivers. The development and implementation of these technologies are creating numerous jobs and stimulating investment, as clearly illustrated by this insightful article on How green energy is driving economic growth. Furthermore, continued innovation in this sector promises further economic expansion and a more sustainable future.
Life Cycle Assessment of Renewable Energy Technologies
Life cycle assessment (LCA) provides a comprehensive framework for evaluating the environmental impacts of renewable energy technologies throughout their entire lifecycle, from raw material extraction to manufacturing, operation, and disposal. A comparative LCA of solar photovoltaic (PV), wind, and hydropower reveals that while all three technologies have lower GHG emissions than fossil fuels, they exhibit different environmental profiles. For example, solar PV’s environmental impact is heavily influenced by the manufacturing process and the disposal of panels, while hydropower’s impact is primarily linked to habitat alteration and water management.
Wind energy, comparatively, tends to have lower impacts across the lifecycle but faces concerns regarding land use and bird mortality.
Carbon Footprint Reduction through Renewable Energy Adoption
The adoption of renewable energy technologies leads to significant carbon footprint reductions. Consider a scenario where a coal-fired power plant with an annual CO2 emission of 1 million tonnes is replaced by a wind farm. Assuming the wind farm has negligible operational emissions and using a methodology that accounts for emissions from manufacturing, transportation, and installation, the carbon footprint reduction would be approximately 1 million tonnes of CO2 per year.
This reduction is calculated by subtracting the emissions associated with the renewable energy technology’s lifecycle from the emissions of the fossil fuel-based alternative. Similar calculations can be performed for other renewable energy technologies, considering their specific emission profiles and the emissions of the replaced energy source. For example, replacing a gasoline-powered vehicle with an electric vehicle charged by solar power would lead to a substantial reduction in the vehicle’s carbon footprint, dependent on the energy mix used to generate the electricity.
The methodology involves a detailed accounting of all emissions associated with each stage of the vehicle’s lifecycle and its energy source.
Final Thoughts
The transition to a sustainable energy future hinges on the continued innovation and deployment of renewable energy technologies. While challenges remain in terms of cost, scalability, and grid integration, the advancements discussed demonstrate a clear path towards a cleaner, more secure, and resilient energy system. Further research and development, coupled with supportive policies and public awareness, are essential to accelerate this vital transformation and secure a brighter energy future for generations to come.
The integration of smart grid technologies and efficient energy storage solutions are pivotal in unlocking the full potential of renewable energy and ensuring a reliable energy supply.
Helpful Answers
What are the main environmental concerns associated with renewable energy technologies?
While generally cleaner than fossil fuels, some renewable technologies have environmental impacts. These include land use (solar farms, wind farms), water consumption (hydropower), and waste generation (solar panel disposal). However, ongoing research focuses on mitigating these impacts through sustainable practices.
How long do different renewable energy technologies last?
Lifespans vary greatly. Solar panels typically last 25-30 years, wind turbines around 20 years, while geothermal plants can operate for decades or even centuries. Battery lifespan depends on the technology and usage, ranging from a few years to over a decade.
What is the role of government policy in promoting renewable energy?
Government policies, including subsidies, tax incentives, and renewable portfolio standards, play a crucial role in driving investment and deployment of renewable energy technologies. These policies help to reduce costs, incentivize innovation, and create a more favorable market environment.
What are the economic benefits of transitioning to renewable energy?
Transitioning to renewable energy creates jobs in manufacturing, installation, maintenance, and research. It reduces dependence on volatile fossil fuel prices, enhances energy security, and can lead to economic growth in related sectors.